Mitigating airborne pathogens indoors: A systematic review of existing and next-generation air cleaning technologies
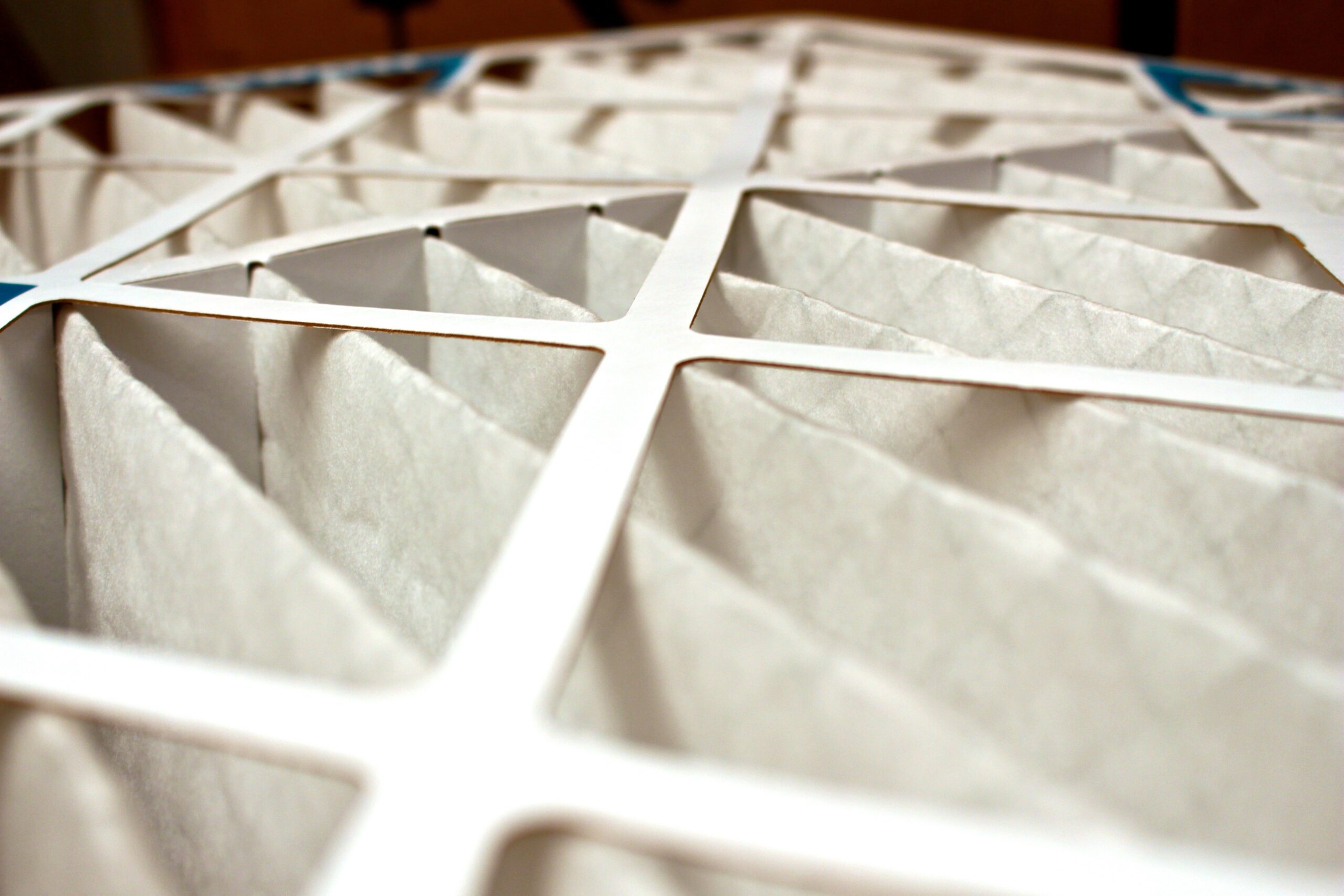
This technical paper provides a systematic review and comparison of existing and next-generation air cleaning technologies.
Abstract
The COVID-19 pandemic has increased global awareness of airborne pathogens and indoor air quality (IAQ), especially in confined spaces where the likelihood of transmission is higher. This systematic review evaluated 14 peer-reviewed studies investigating indoor air treatment technologies, including ultraviolet (UV) systems, filtration, ionisation, and hybrid approaches. UVC-mirror system demonstrated the highest pathogen removal efficacy (up to 99.99%) with minimal maintenance and without generating harmful by-products. However, these technologies do not distinguish between harmful and beneficial microorganisms, potentially disrupting microbial diversity and reducing exposures that contribute to overall health. In contrast, indirect evaporative cooling (IDEC) systems reduce airborne infection risk through passive mechanisms by enhancing outdoor air exchange and aerosol dispersion, while preserving microbial diversity. IDEC also operates in an energy-efficient manner with added benefits of humidity control. This review underscores the importance of integrating active and passive strategies to manage IAQ, supporting infection control while maintaining ecological balance in the indoor microbiome.
Introduction
Following the COVID-19 pandemic, public awareness of airborne pathogens and indoor air quality (IAQ) has surged, driving demand for residential and commercial air cleaning technologies. IAQ is increasingly recognised as a critical factor influencing occupant health, productivity, comfort, and wellbeing1, 2. People spend over 90% of their time indoors or in air conditioned vehicles3. In enclosed residential or occupational settings, susceptible individuals can be repeatedly exposed to contagious bioaerosols. As a result, the type of ventilation system significantly influences exposure levels and potential adverse health effects. Systems with low air changes per hour (ACH), such as some air conditioning (AC) units, have been linked to increased disease risk due to continuous recirculation of the same air.
Researchers have identified that approximately 14% of the genes detected in AC environments are associated with neurodegenerative, cancer-related, viral, and bacterial infectious diseases. This association is attributed to the presence of pathogens including Mycobacterium tuberculosis, Acinetobacter baumannii, Staphylococcus aureus, Enterococcus faecalis, and viruses including HSV, HPV, HTLV-1, HIV, and influenza A4.
In response, numerous air cleaning technologies have been developed to reduce airborne transmission risks. Most UVC disinfection and filtration technologies are designed for broad-spectrum microbial inactivation. These systems rely on physical or chemical mechanisms, including ultraviolet-induced nucleic acid damage or reactive oxygen species, to eliminate both pathogenic and non-pathogenic organisms. While effective in reducing microbial load, these approaches may also disrupt the microbial ecology of indoor environments and reduce beneficial microbial exposures that support immune tolerance.
Among emerging alternatives, indirect evaporative cooling (IDEC) systems offer a passive and non-destructive method for reducing airborne infection risk. IDEC systems operate through enhanced outdoor air exchange and air mixing – mechanisms that dilute and disperse bioaerosols away from their source and reduce infectivity without directly inactivating microorganisms. This distinguishes IDEC from conventional disinfection systems: while most favour non-selective microbial elimination, IDEC supports a more balanced approach, reducing exposure while potentially preserving microbial diversity. This background is necessary to understand the relevance of maintaining microbial diversity, especially when considering the benefits of non-pathogens described below.
Historical evidence reinforces the need for air cleaning solutions that are effective yet ecologically balanced. Airborne pathogens have caused numerous infectious diseases, some leading to pandemics, as outlined in table 1. These include tuberculosis (Mycobacterium tuberculosis), measles and mumps (paramyxoviruses), whooping cough (Bordetella pertussis), and aspergillosis (Aspergillus species). COVID-19 (SARS-CoV-2) resulted in a global pandemic, contributing to approximately 25,000 deaths in Australia and over 7.1 million deaths worldwide5. One example is a 1992 tuberculosis outbreak in a Minnesota bar, where 41 of 97 patrons tested positive and 14 developed active infection despite a typical transmission rate of only 1–2% among close contacts6.
Outdoor and indoor air pollution stresses our respiratory system and may increase susceptibility to viral infections. Exposure to particulate matter and ozone-induced oxidative stress has been linked to increased severity and mortality following respiratory viral infections7, 8. As urbanisation continues to rise, managing airborne transmission in indoor environments is increasingly essential.
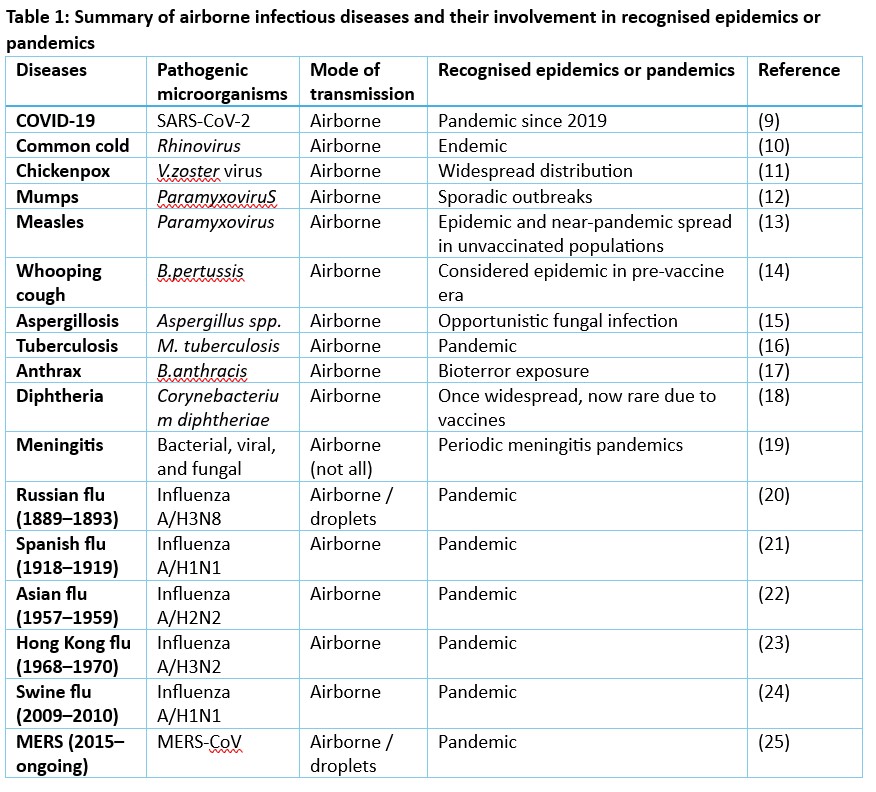
Indoor airborne microorganisms
Non-pathogenic microorganisms
Non-pathogenic microbes, including species found in indoor and airborne environments, play a critical role in human health26. Breathing in these microbes, often aerosolised from dust or present in normal indoor settings, can modulate immune responses, as seen with Acinetobacter lwoffii’s anti-allergy effects27. Probiotics like Bifidobacterium longum and Lactobacillus sakei are common indoors, and further support gut and sinus health when inhaled in low doses, potentially reducing inflammation28. However, for sensitive, vulnerable, immunocompromised, or sick individuals, these typically beneficial microbes can pose risks. Inhaled in high concentrations, even non-pathogenic species may overwhelm weakened immune systems, potentially causing opportunistic infections or exacerbating conditions like asthma, particularly in hospital settings where Bacillus spores or Enterococcus from dust could proliferate29.
Air microbes: Acinetobacter lwoffii, Bacillus subtilis, and Mycobacterium vaccae are commonly detected in ambient air and have been linked to immune regulation, gut health, stress resilience, and protection against neurodegenerative conditions30.
Indoor microbes: Lactobacillus fermentum, Propionibacterium freudenreichii, and Lactobacillus helveticus are frequently found in indoor environments and are associated with cholesterol reduction, obesity management, and anti-allergy effects30.
Dust-aerosolised: Propionibacterium freudenreichii and Clostridium histolyticum have been identified in indoor dust and are reported to contribute to cancer prevention, metabolic health, and treatment of Peyronie’s disease30.
Human health impacts
Health impacts were extracted from the “Health Effect” column in the Tableau database of beneficial microbes30.
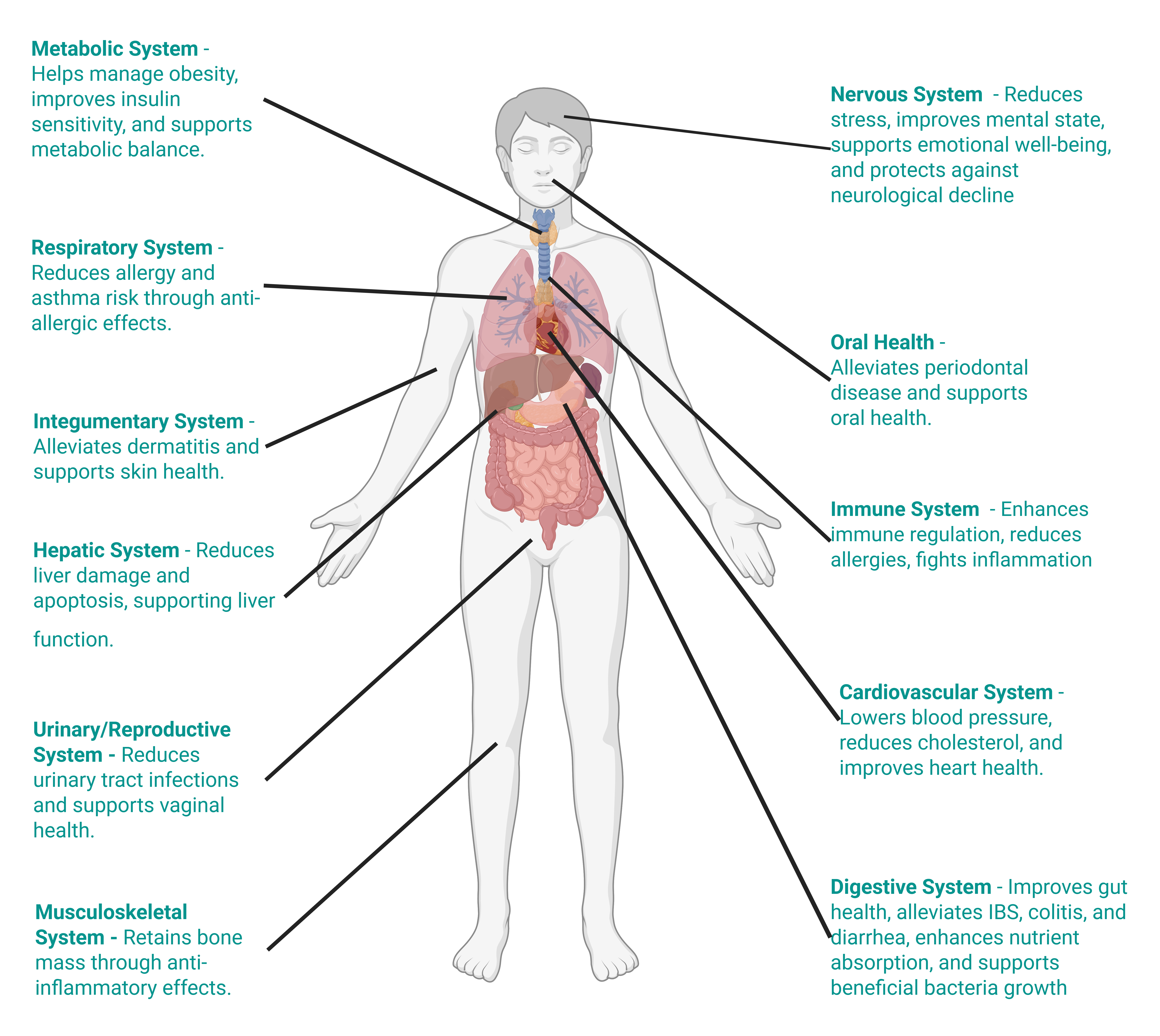
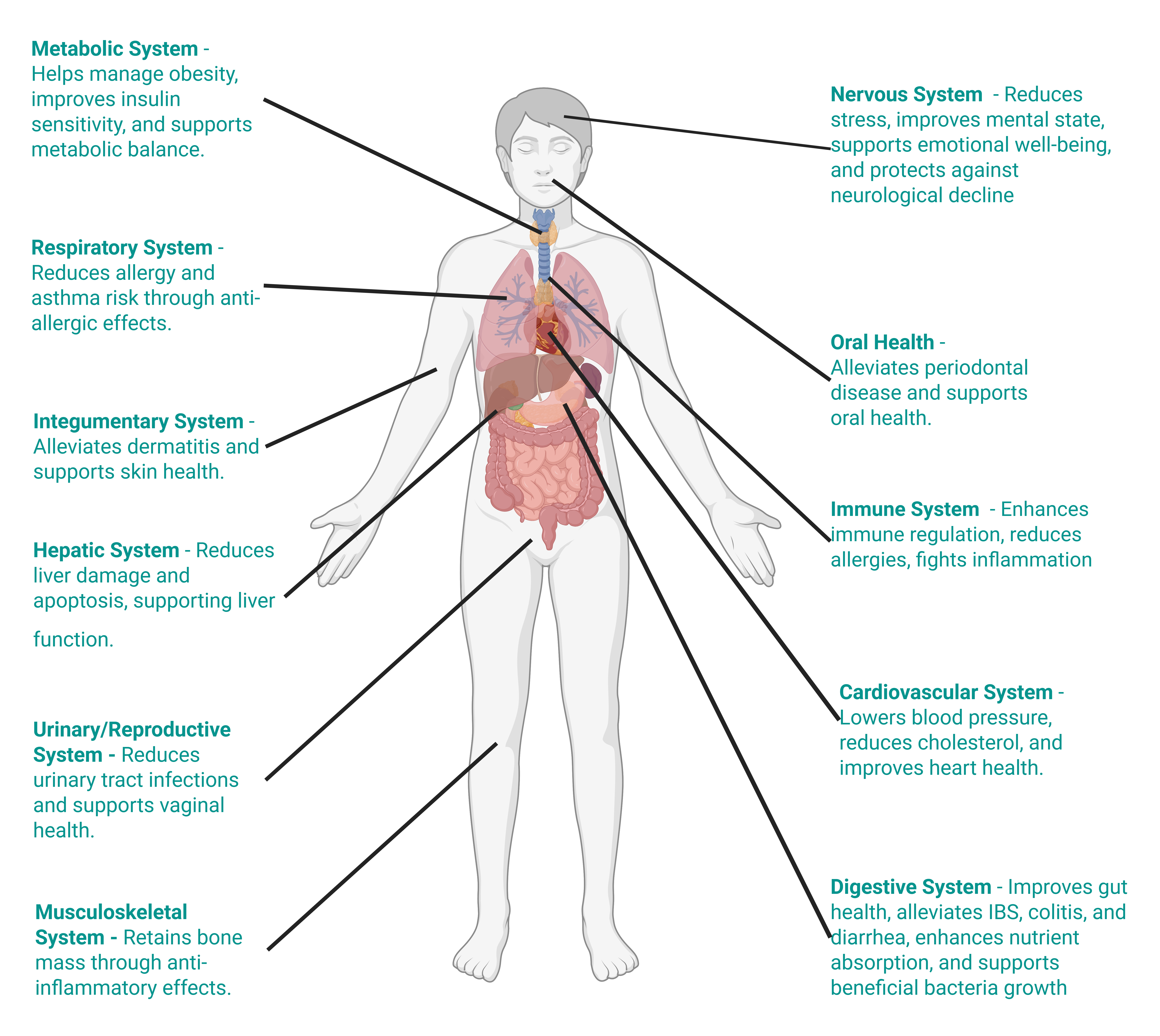
Indoor airborne pathogenic microorganisms
Many airborne pathogens affect multiple body systems, with their impacts varying by type and mode of exposure. Airborne transmission has been shown to be a major public health threat that caused mortality in the pre-vaccination era31. Some airborne pathogens – particularly in occupational settings such as agriculture or food processing – act as respiratory allergens rather than infectious agents. When inhaled, they may trigger immune responses like asthma, allergic rhinitis, or hypersensitivity pneumonitis32. Unlike infections, these conditions result from immune sensitisation rather than direct microbial invasion, leading to infectious disease33. From a numerical point of view, bacteria-like particle and viral-like particle bioaerosol concentrations are around 4.8 × 105 , 5.2 × 105 particles/m3 in an indoor setting34. To reduce airborne pathogens, and to render them non-infectious/non-allergenic for susceptible healthy individuals, technologies based on inactivation/removal/dilution should be focused.
Modes of transmission
Transmission of respiratory pathogens occurs via direct or indirect contact, inhalation of droplets or aerosols, or a combination of these routes. These are expelled during natural respiratory activities such as exhaling, talking, sneezing, coughing, laughing, or singing. Artificial aerosol generation occurs through activities like toilet flushing, running taps, HVAC systems, evaporative cooling, and medical procedures (e.g., intubation, and surgery). Once released, they may settle due to gravity (short-range transmission), be carried by air currents, or remain airborne (long-range transmission) before inhalation by a susceptible individual. At short range, both contact and inhalation pose risks; at longer distances, once large droplets (>100 µm) settle, inhalation predominates. Aerosols may also form via the resuspension of settled dust particles. Bioaerosols may contain viruses, bacteria, fungi, cellular debris, toxins, and electrolytes from respiratory fluids. They vary in size, with viruses ranging from 0.017–0.3µm and most fungal particles over 1µm. Transmission risk is influenced by aerosol generation rates, respiratory deposition site, and infectious doses. Environmental factors such as temperature, humidity, ventilation, UV radiation, and air exchange rates affect survival and dispersal. Viral characteristics (e.g., envelope structure, stability) and host factors (e.g., immunity, age) also shape transmission dynamics35.
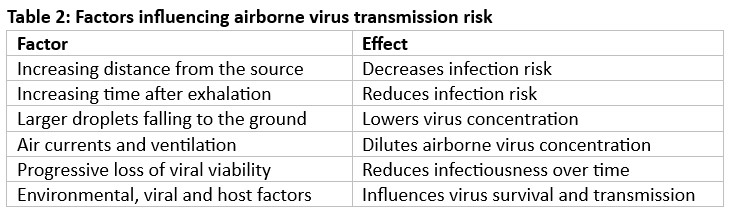
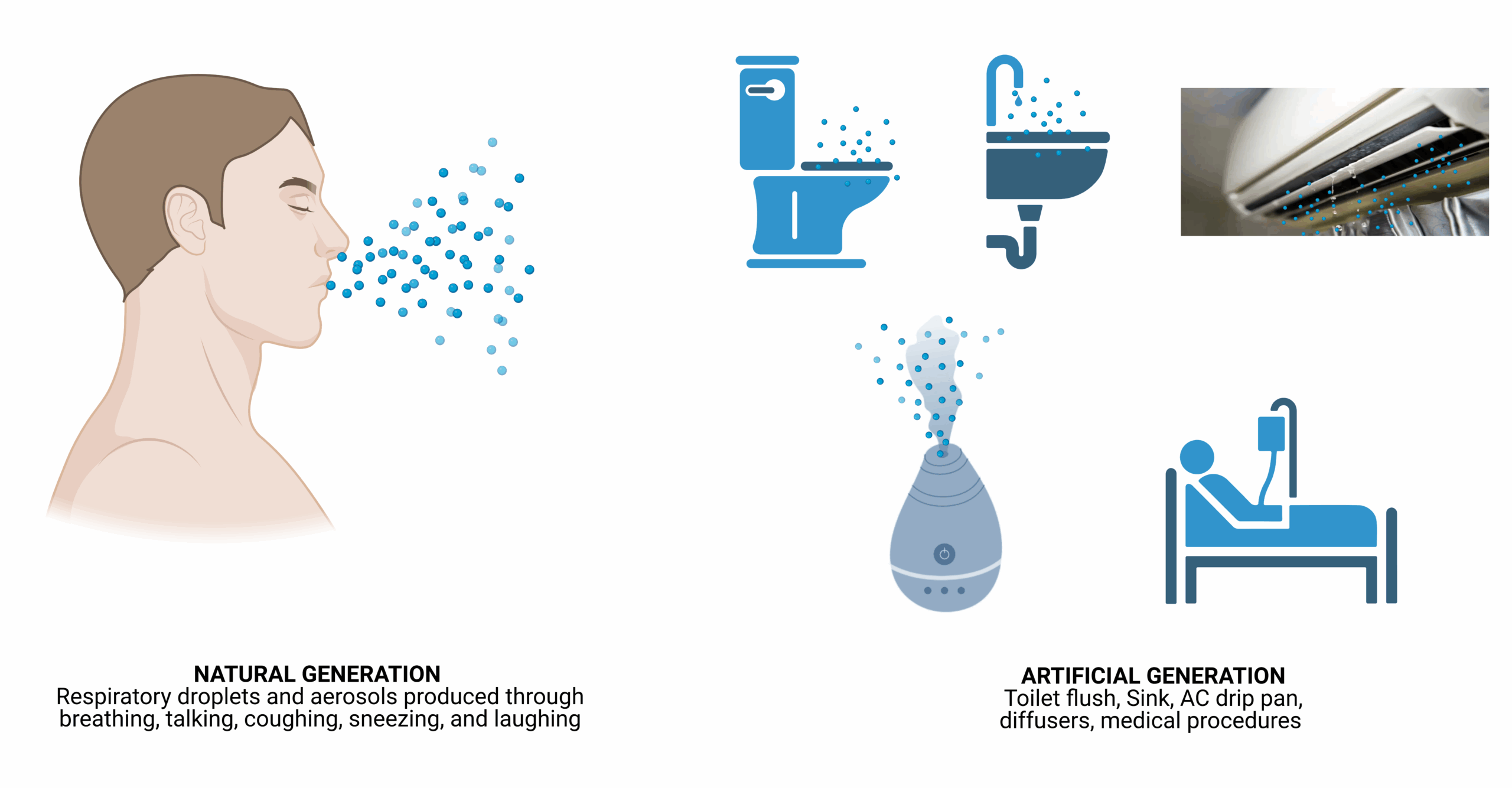
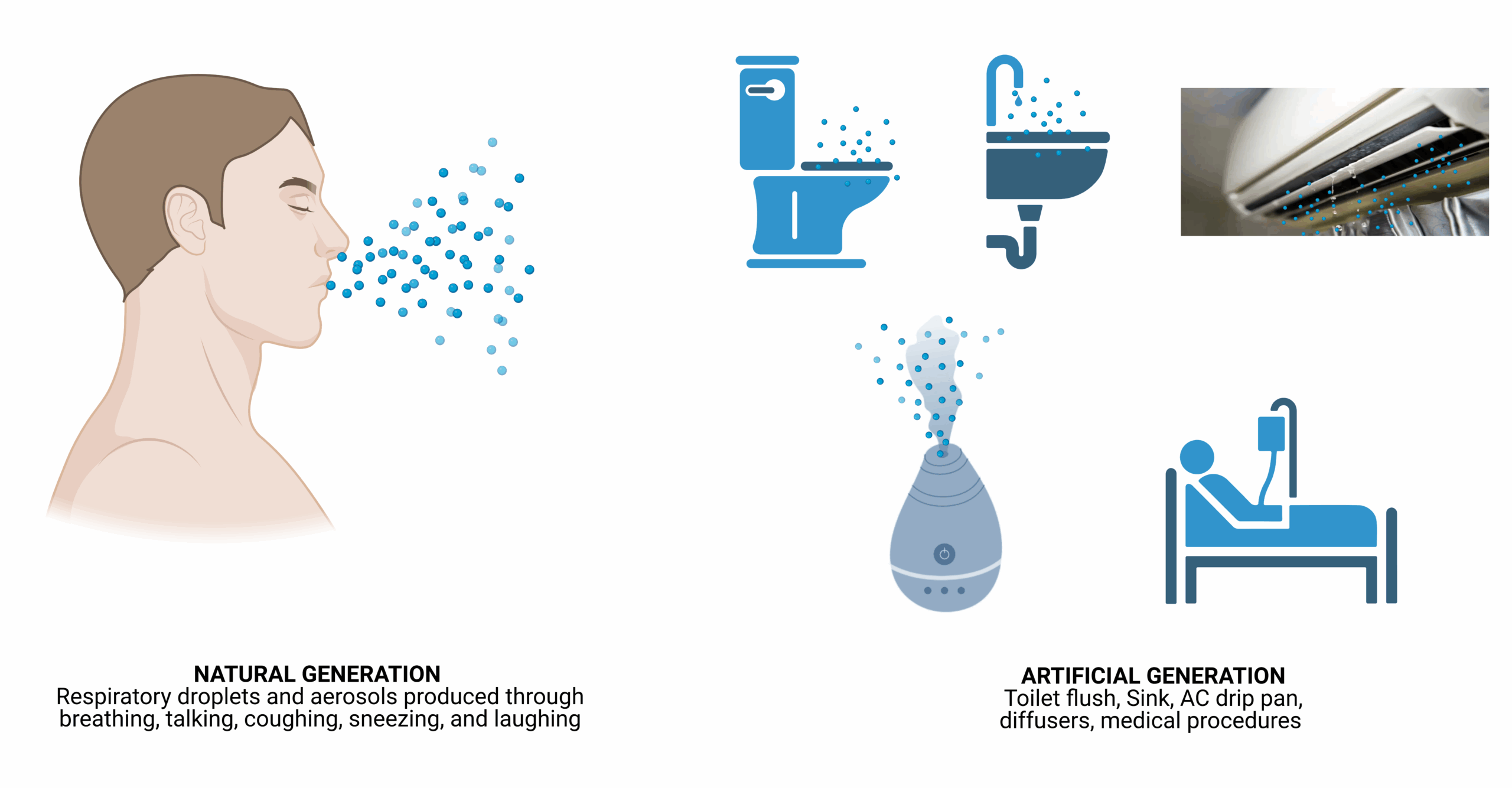
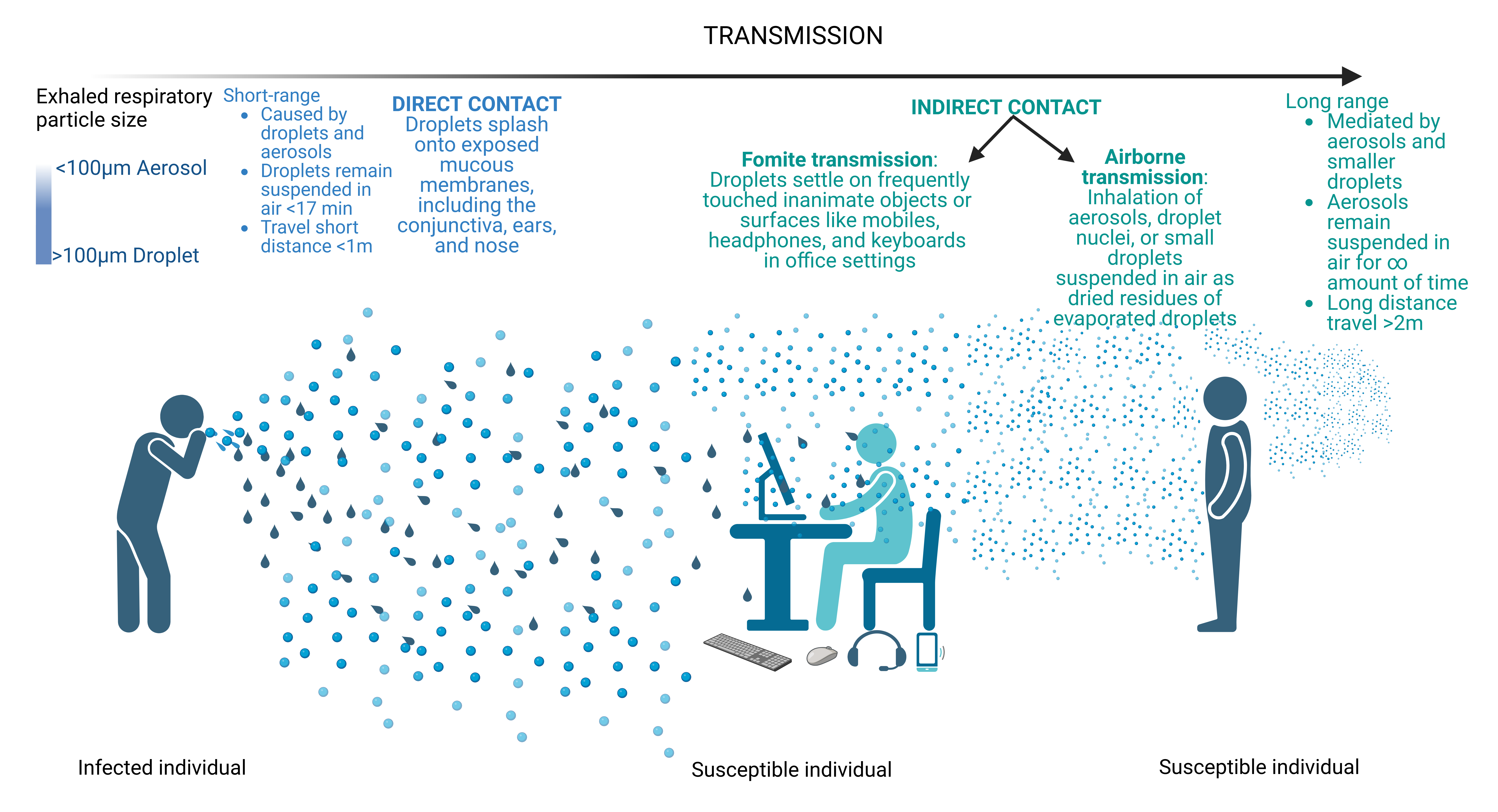
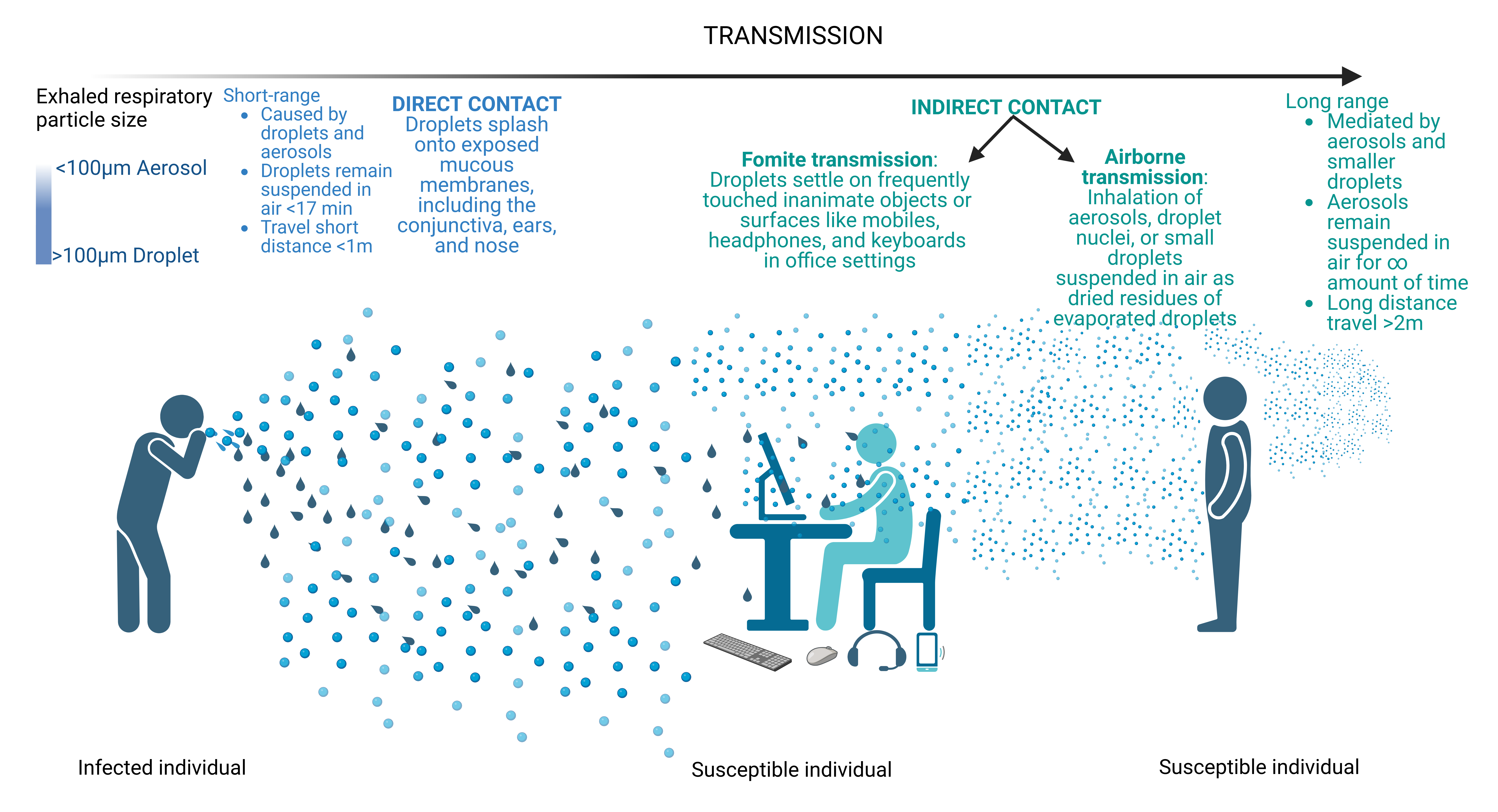
Methods
The Scopus database was searched using the terms “indoor air” OR “indoor environment” OR “indoor air quality” OR “IAQ” combined with “pathogen removal” OR “pathogen inactivation”, resulting in 14 identified articles. Screening was conducted as outlined in Figure 1. Abstracts of the selected articles were included in the final analysis.
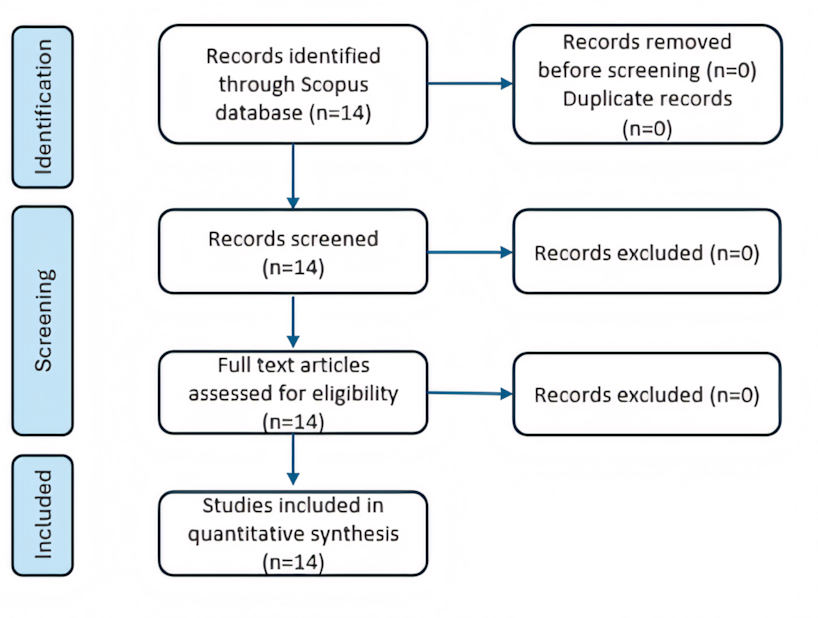
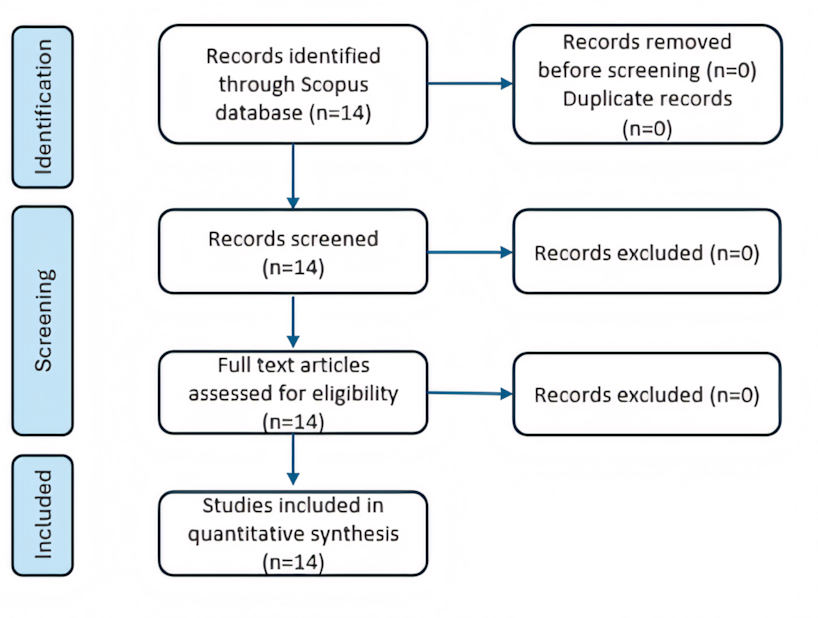
Results
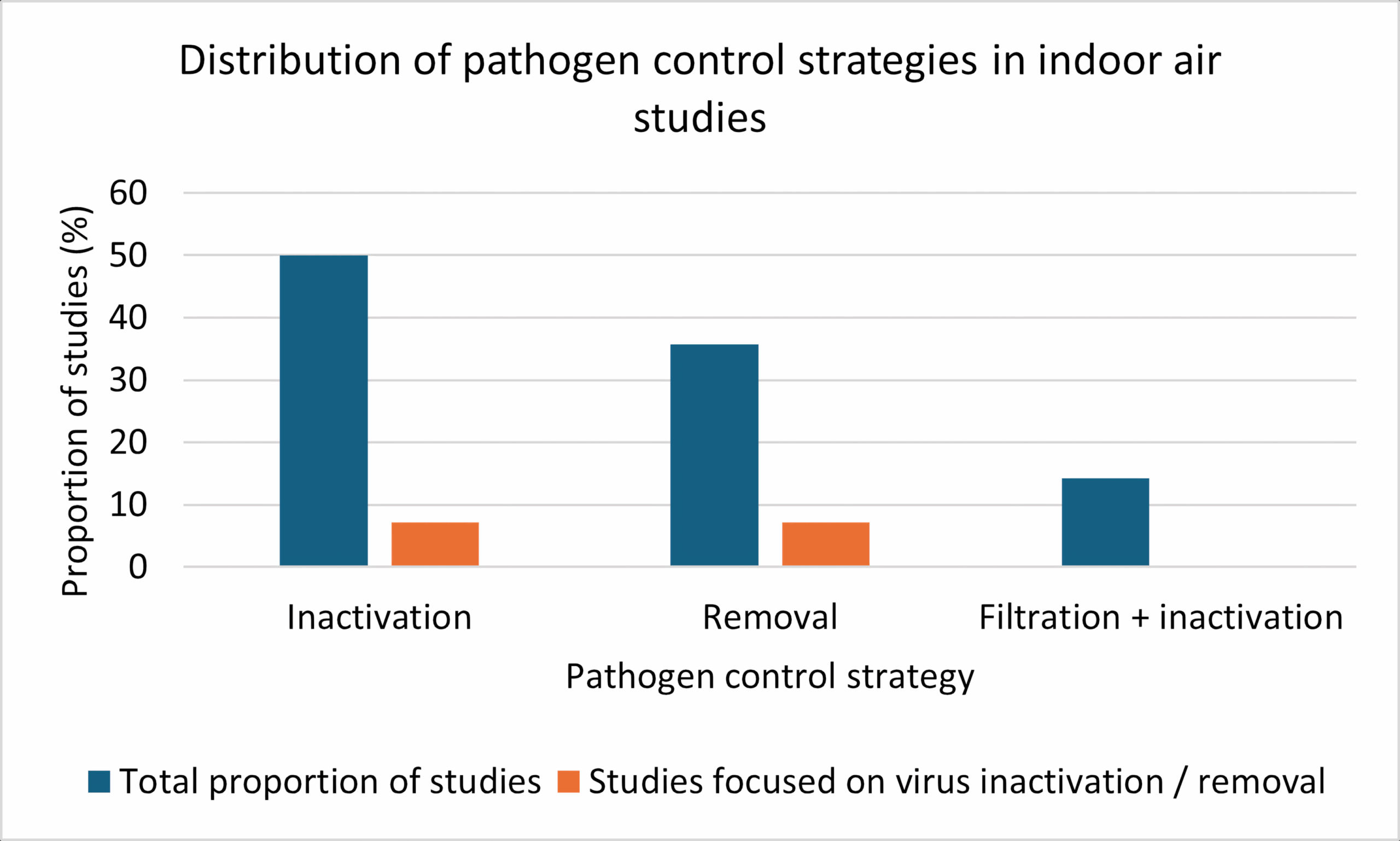
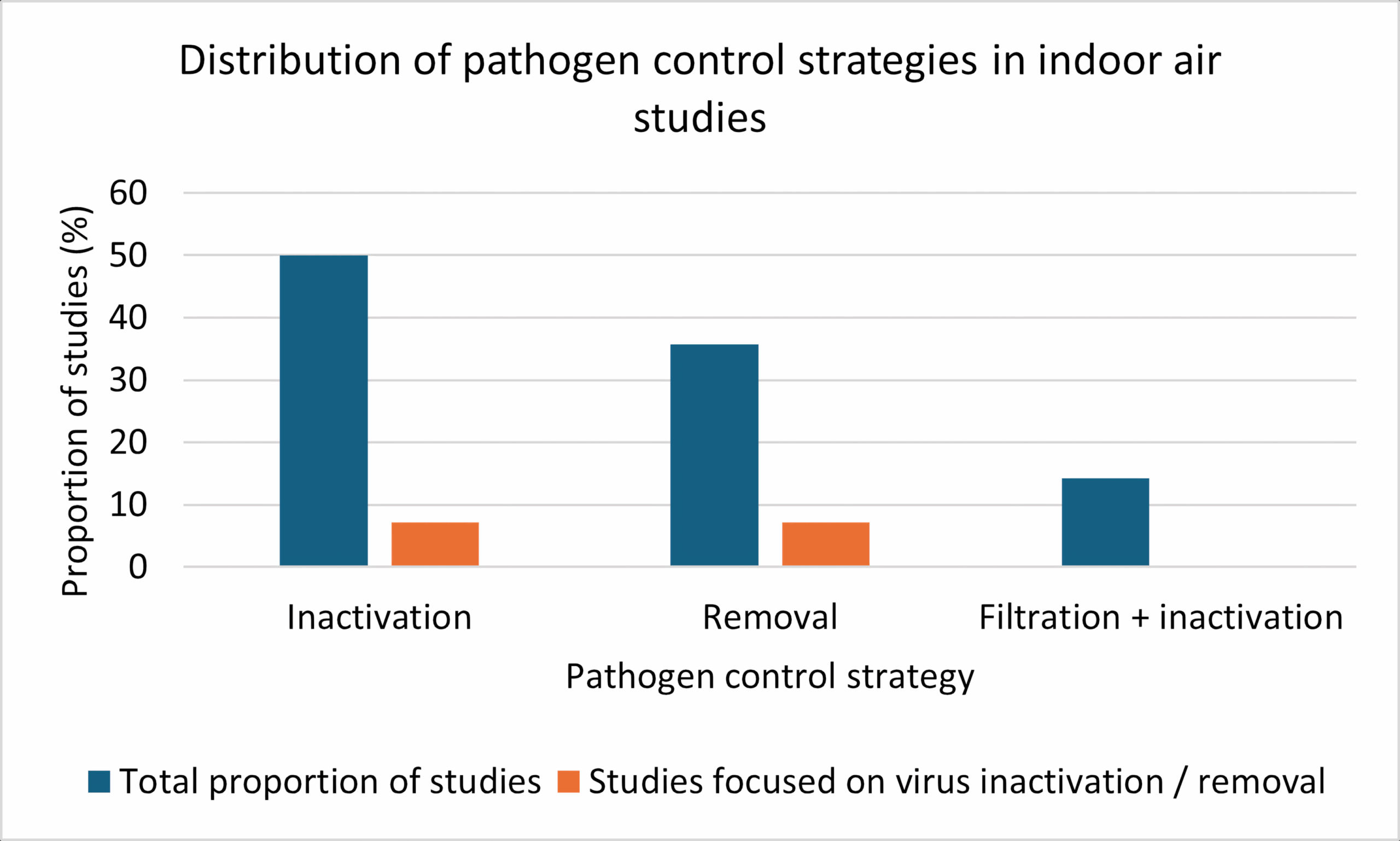
A total of 14 peer-reviewed studies were evaluated to determine the primary objective of pathogen control technologies used for indoor air disinfection. As shown in Figure 4, inactivation emerged as the dominant strategy, reported in 50% of studies (n=7). Removal-based technologies were applied in 35% of studies (n=5) while systems combining both filtration and inactivation accounted for 15% (n=2). Only a small fraction of studies (7%) specifically focused on virus inactivation or virus removal, respectively. These results indicate that most mitigation strategies target broad-spectrum microbial inactivation over virus-specific approaches. Figure 5 presents the proportional distribution of the different pathogen control strategies among the included indoor air treatment studies.
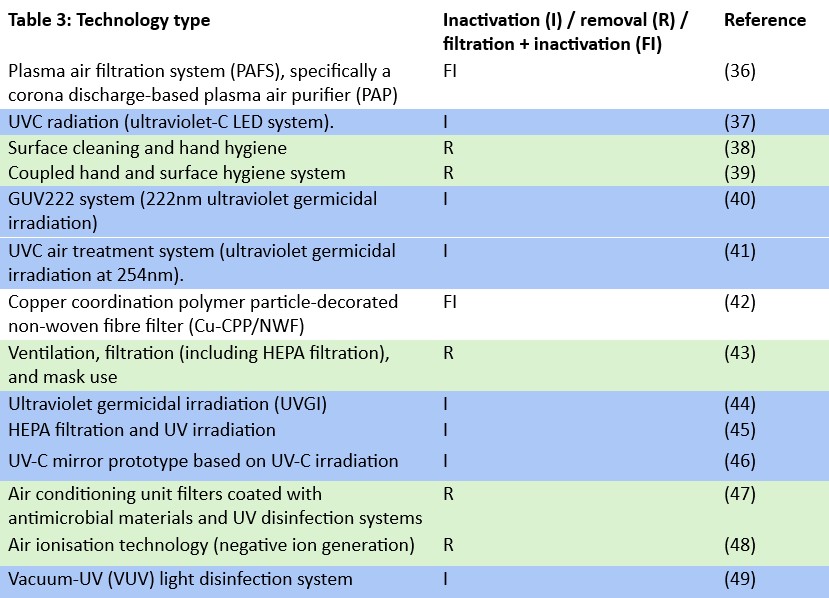
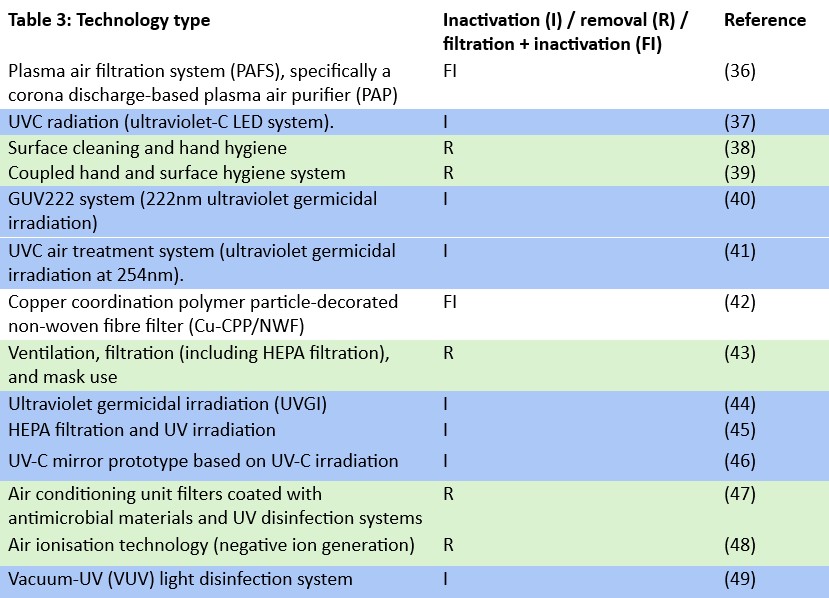
Inactivation
All seven studies employed ultraviolet-based systems, including ultraviolet C radiation (UV-C 254nm), far-UVC (222nm), and vacuum UV (185nm), targeting airborne pathogens via DNA/RNA damage or oxidative stress37, 40, 41, 44-46. UVC systems disrupted genetic material, preventing microbial replication37, 41, 44-46, while VUV-induced reactive oxygen species (ROS) and ozone further contributed to pathogen inactivation49. Most systems achieved rapid inactivation (<1–10 min), with log reductions ranging from 3–6-log41, 44, 49. Only two systems reported co-benefits for particle or volatile organic compound (VOC) oxidation40, 49. By-product generation was noted in systems using 222nm and 185nm, primarily ozone and secondary organic aerosol (SOA)40, 49, whereas UVC systems (254nm) did not report harmful residues37, 41, 44-46. Advantages included high inactivation efficacy, compact design, and integration potential with HVAC44-46. Disadvantages involved ozone toxicity risks40, 49 and reduced performance against UV-resistant pathogens46. Ideal application settings included hospitals, offices, ventilation ducts, and small enclosed rooms. Systems functioned effectively under controlled humidity and moderate airflow, with optimal performance at 18–25 °C and RH ~50%36, 41, 44, 46, 49.
Removal
The reviewed studies focused on removal-based indoor air disinfection strategies, including surface hygiene38, 39, ventilation and filtration43, antimicrobial HVAC filters with UV47, and ion-enhanced deposition48. These technologies removed pathogens through physical mechanisms such as disinfection of fomites, dilution and capture of aerosols, or enhanced particle deposition via electrostatic charge. Surface cleaning and hand hygiene showed significant reductions in surface contamination within 70 minutes, though were ineffective when applied in isolation. Ventilation and HEPA filtration effectively removed airborne pathogens and also addressed other co-occurring indoor pollutants such as CO₂ and particulate matter (PM), achieving up to 99% filtration efficiency. Antimicrobial filters combined with UV offered rapid disinfection (<2 minutes) but raised concerns about potential microbial resistance. Ionisation increased deposition rates by up to 650% for virus-sized particles, with effectiveness dependent on airflow direction and ion concentration. Importantly, none of these methods produced harmful by-products, with the exception of biocidal surface treatments, which may contribute to the development of resistant organisms. These systems were most effective under controlled indoor conditions (specifically temperatures between 20–25 °C and relative humidity levels of 40–60%) and were applicable to diverse settings such as hospitals, offices, schools, and transport. Overall, removal-based technologies provided complementary protection to inactivation systems, with their success contingent on consistent use, strategic placement, and environmental HVAC control.
Filtration-inactivation hybrid
The plasma air filtration systems (PAFS) and copper coordination polymer particle-decorated non-woven fibre filters (Cu-CPP/NWF) evaluated integrated with pathogen inactivation mechanisms, achieving dual control of bioaerosols and chemical pollutants36, 42. The PAFS used corona discharge and Fe-Ni metal foam to trap and inactivate aerosols, employing reactive oxygen and nitrogen species (RONS) such as OH, HOONO, and H₂O₂ for cell lysis36. Similarly, the Cu-CPP/NWF system inactivated bacteria via Cu²⁺-induced membrane disruption and ROS production42. Both systems were highly effective, with reported pathogen reductions of ≥99.3% and 100% for E. coli respectively36, 42. These technologies also demonstrated strong particulate matter removal (≥90.9% PM36 and 99.99% PM2.542. VOC (e.g., p-xylene) and CO₂ adsorption were an added benefit of the copper-based filter that achieved full disinfection within 45 minutes. In comparison, one hour is required for effective inactivation due to the additional filters used to minimise ozone and NOx by-products from plasma discharge36, 42. Advantages included reusable modular components, high removal efficiency, and integration potential with HVAC systems. However, PAFS risk low-level ozone generation, while the Cu-CPP/NWF’s performance declines under high humidity. Both systems operated optimally under standard indoor conditions (20–25°C, 40–55% RH) and are suitable for enclosed public environments, hospitals, and offices36, 42.
Highest removal rate
Among the evaluated technologies, ultraviolet-based systems demonstrated the highest pathogen inactivation, reporting up to 99.99% bacterial and 90% viral reduction using a UVC-mirror system44. Similarly, the ultraviolet-C LED system and UVC air treatment system (254nm) achieved over 99% inactivation with UVC filters and up to 6.48-log₁₀ reduction for MS2 coliphage, respectively38, 42. The VUV system showed ≥6-log₁₀ reductions in E. coli and 5-log₁₀ for Mycobacterium tuberculosis using vacuum UV, while Cu-CPP/NWF reported 100% E. coli inactivation with copper-based filters42. Moderate efficacy was observed in hybrid systems such as HEPA filtration and UV irradiation, which recorded 3-log₁₀ reductions in under 11 minutes45. Ion-assisted deposition improved removal by up to 654%, and manual hygiene strategies showed strong surface decontamination37, 39. Lower-performing studies, including GUV 222 system UVGI, lacked quantitative metrics or raised concerns around by-product formation40, 44. Overall, UVC-based interventions, particularly duct-integrated systems, offer the most reliable and practical solution for airborne pathogen control in indoor environments.
Long-term feasibility
Among the technologies reviewed, the UVC-mirror system stands out as the most suitable for long-term, low-maintenance use. It operates passively once installed, requiring only UV lamp replacement every 8,000–10,000 hours and no regular cleaning. Its reflective cavity design enhances inactivation efficiency while protective UV-transparent glass minimises fouling46. In contrast, other UV systems demand regular maintenance, careful lamp monitoring, or system calibration to avoid ozone generation or exposure risks37, 40, 41, 44. Filtration-based and plasma systems require regular cleaning, component monitoring, or replacement, which increases long-term upkeep. Behavioural strategies, while important during outbreaks, rely heavily on compliance and do not offer consistent automated control37-39, 42, 43. Therefore, the UVC-mirror’s minimal intervention requirement, combined with effective airborne pathogen inactivation, makes it the most practical choice for sustained application.
Evaluating trade-offs: Efficacy, risks, suitability, and ventilation integration
Among the technologies reviewed, the UVC-mirror system offers the most practical and effective long-term solution for indoor air disinfection. It achieves high pathogen inactivation (up to 99.99 %) and does not produce harmful by-products46. Designed for integration within HVAC ducts, it operates efficiently under standard temperature and humidity conditions without disrupting airflow. In contrast, while plasma filtration and VUV systems are effective, they generate ozone, posing potential health risks36, 49. Manual hygiene interventions are reliant on user compliance and not suited to airborne control. Although single-pass UVC is highly effective, it demands warm-up time and close environmental/operational control41. Copper-based filters show promise but perform poorly in humid conditions42. Ion-based systems enhance deposition but lack pathogen inactivation48. Overall, the UVC-mirror system provides the best balance of efficacy, safety, and ease of operation for sustained indoor air quality management.
Timeframe for inactivation
When prioritising rapid microbial inactivation, the ultraviolet-C LED system demonstrates the fastest response, achieving over 99% pathogen reduction within approximately 24ms37. This far outpaces other systems, including the UVC-mirror system which, although highly effective and low-maintenance, requires under one second exposure46. Similarly, single-pass UVC systems reach complete inactivation in less than one second, but need 30 minutes of warm-up before effective operation, reducing their practical responsiveness41. Systems like plasma filtration and copper-based filters achieve strong microbial reduction but require up to 45–60 minutes for full effect36, 42. Manual hygiene strategies and ion-enhanced deposition take tens of minutes to reach steady control, making them less suitable when immediate air disinfection is required37, 39, 38. While several technologies are effective, UV-C clearly leads in speed, making it best suited for high-risk, time-critical environments such as hospitals or rapid-response containment areas.
Discussion
Targeted mitigation vs IDEC-based passive mechanism
Among the technologies reviewed, none explicitly aim to safeguard non-pathogenic airborne microorganisms. Most systems – such as UVC disinfection, plasma air filtration, and copper-based antimicrobial filters – are designed for broad-spectrum microbial inactivation. These methods rely on direct physical or chemical mechanisms, including ultraviolet-induced nucleic acid damage or reactive oxygen species, which effectively eliminate both pathogenic and non-pathogenic organisms. While these methods enhance disinfection efficacy, they may inadvertently disrupt the microbial ecology of indoor environments and reduce the beneficial microbial exposures that support immune tolerance.
In contrast, indirect evaporative cooling (IDEC) systems function through passive mechanisms, including the introduction of large volumes of outdoor air, enhanced air mixing, and promoting droplet evaporation. The processes by which evaporative cooling dilutes bioaerosols include high air exchange rates (20–30 ACH), creation of positive indoor pressure, filtration through media pads, shortened residence time of indoor pollutants, and the absence of air recirculation – all of which contribute to the physical removal and dispersion of airborne microorganisms50-53. These processes dilute bioaerosols, disperse particles away from their source, and reduce particle size, thereby lowering the infectivity of viruses and reducing occupant exposure risk. Notably, IDEC does not directly inactivate microorganisms, thereby avoiding collateral impacts on beneficial microbiota. This mode of action may support healthier indoor environments by preserving microbial diversity while still reducing infection risk through physical removal pathways. Therefore, IDEC represents a distinct approach among air treatment strategies by mitigating pathogen exposure without compromising the microbial balance of the built environment.
Conclusion
This systematic review highlights the technologies available for mitigating airborne pathogens in indoor environments, with ultraviolet-based systems. While most technologies prioritise broad-spectrum microbial inactivation through direct physical or chemical mechanisms, none consider the ecological implications of indiscriminately eliminating both harmful and beneficial microorganisms. In contrast, IDEC presents a passive yet effective alternative by promoting dilution, dispersion, and evaporation, thereby lowering pathogen exposure without disrupting microbial diversity.
The UVC-mirror system demonstrated the most balanced performance across efficacy, operational simplicity, safety, and HVAC compatibility, positioning it as the most practical long-term solution. However, technology selection must be context- and parameter-specific, considering the pathogen load, building design, occupant vulnerability, and maintenance capacity. Future strategies should incorporate an understanding of the indoor microbiome, recognising the potential health benefits of preserving non-pathogenic organisms. Integrating passive and active approaches – rather than relying solely on pathogen-targeted systems – may offer a more sustainable and holistic pathway for managing IAQ and protecting public health.
Author information
Corresponding author
Sonali Shrikant Deshmukh – Seeley International Pty. Ltd.; College of Science and Engineering, Flinders University, Bedford Park, SA 5042, Australia; The Aerobiome Innovation and Research Hub, Flinders University, Bedford Park, SA 5042, Australia; ORCID iD: 0009-0005-7234-398X;
Email: [email protected]
Authors
Clinton Leonardis – Seeley International Pty. Ltd. ORCID iD: 0009-0005-9698-9531
Parick McCaffrey – Seeley International Pty. Ltd. ORCID iD: 0009-0007-3081-5672
Emma Kuhn – College of Science and Engineering, Flinders University, Bedford Park, SA 5042, Australia; The Aerobiome Innovation and Research Hub, Flinders University, Bedford Park, SA 5042, Australia; ORCID iD: 0000-0002-1152-1851
Author contributions
SSD performed the literature search and evaluated the titles and abstracts of each article independently. SSD developed the first manuscript draft. CL, PMcC, and EK supervised the process and reviewed the manuscript. All the authors contributed to the interpretation of the data and read and approved the final manuscript. All authors have read and agreed to the published version of the manuscript.
References
1. Mujan I, Anđelković AS, Munćan V, Kljajić M, Ružić D. Influence of indoor environmental quality on human health and productivity-A review. Journal of cleaner production. 2019;217:646-57.
2. Arif M, Katafygiotou M, Mazroei A, Kaushik A, Elsarrag E. Impact of indoor environmental quality on occupant well-being and comfort: A review of the literature. International Journal of Sustainable Built Environment. 2016;5(1):1-11.
3. Pruszyński J, Cianciara D, Włodarczyk-Pruszyńska I, Górczak M, Padzińska-Pruszyńska I. Indoor Generation Era. Risks and challenges. Journal of Education, Health and Sport. 2023;48(1):23-40.
4. Liu M, Shen B, Gan Z, Liu J, Li Q, Ma T, et al. Operation of air conditioners affects the microbial pathogenic potential in bedrooms: A metagenomics and culture-dependent study. Building and Environment. 2023;239:110335.
5. Organization WH. WHO COVID-19 dashboard [Available from: https://data.who.int/dashboards/covid19/deaths?n=o.
6. Kline SE, Hedemark LL, Davies SF. Outbreak of tuberculosis among regular patrons of a neighborhood bar. New England Journal of Medicine. 1995;333(4):222-7.
7. Domingo JL, Rovira J. Effects of air pollutants on the transmission and severity of respiratory viral infections. Environmental research. 2020;187:109650.
8. Kesic MJ, Meyer M, Bauer R, Jaspers I. Exposure to ozone modulates human airway protease/antiprotease balance contributing to increased influenza A infection. PloS one. 2012;7(4):e35108.
9. WHO. WHO COVID-19 dashboard: World Health Organization;
10. Piret J, Boivin G. Pandemics throughout history. Frontiers in microbiology. 2021;11:631736.
11. Gershon AA, Breuer J, Cohen JI, Cohrs RJ, Gershon MD, Gilden D, et al. Varicella zoster virus infection. Nature reviews Disease primers. 2015;1(1):1-18.
12. Gouma S, Koopmans MP, van Binnendijk RS. Mumps virus pathogenesis: insights and knowledge gaps. Human vaccines & immunotherapeutics. 2016;12(12):3110-2.
13. Rota PA, Moss WJ, Takeda M, de Swart RL, Thompson KM, Goodson JL. Measles. Nature Reviews Disease Primers. 2016;2(1):16049.
14. Warfel JM, Beren J, Merkel TJ. Airborne transmission of Bordetella pertussis. J Infect Dis. 2012;206(6):902-6.
15. Dagenais TR, Keller NP. Pathogenesis of Aspergillus fumigatus in Invasive Aspergillosis. Clin Microbiol Rev. 2009;22(3):447-65.
16. Coleman M, Martinez L, Theron G, Wood R, Marais B. Mycobacterium tuberculosis Transmission in High-Incidence Settings-New Paradigms and Insights. Pathogens. 2022;11(11).
17. Fennelly KP, Davidow AL, Miller SL, Connell N, Ellner JJ. Airborne infection with Bacillus anthracis–from mills to mail. Emerg Infect Dis. 2004;10(6):996-1002.
18. Meredith T. Chapter 133-Vitrectomy for infectious endophthalmitis. Retina (Fourth Edition) Edinburgh: Mosby. 2006:2255-75.
19. Meningitis [1 April 2025]. Available from:
20. Sun H, Li H, Tong Q, Han Q, Liu J, Yu H, et al. Airborne transmission of human-isolated avian H3N8 influenza virus between ferrets. Cell. 2023;186(19):4074-84.e11.
21. Siddiqui. TNJRTJADNAH. H1N1 Influenza.
22. Kutter JS, Linster M, de Meulder D, Bestebroer TM, Lexmond P, Rosu ME, et al. Continued adaptation of A/H2N2 viruses during pandemic circulation in humans. J Gen Virol. 2023;104(8).
23. Cowling BJ, Ip DK, Fang VJ, Suntarattiwong P, Olsen SJ, Levy J, et al. Aerosol transmission is an important mode of influenza A virus spread. Nat Commun. 2013;4:1935.
24. Zhang H, Li X, Ma R, Li X, Zhou Y, Dong H, et al. Airborne spread and infection of a novel swine-origin influenza A (H1N1) virus. Virol J. 2013;10:204.
25. Kim SH, Chang SY, Sung M, Park JH, Bin Kim H, Lee H, et al. Extensive Viable Middle East Respiratory Syndrome (MERS) Coronavirus Contamination in Air and Surrounding Environment in MERS Isolation Wards. Clin Infect Dis. 2016;63(3):363-9.
26. Strachan DP. Hay fever, hygiene, and household size. Bmj. 1989;299(6710):1259-60.
27. Debarry J, Hanuszkiewicz A, Stein K, Holst O, Heine H. The allergy‐protective properties of Acinetobacter lwoffii F78 are imparted by its lipopolysaccharide. Allergy. 2010;65(6):690-7.
28. Yao S, Zhao Z, Wang W, Liu X. Bifidobacterium Longum: Protection against Inflammatory Bowel Disease. J Immunol Res. 2021;2021:8030297.
29. Wenzel SE. Asthma phenotypes: the evolution from clinical to molecular approaches. Nat Med. 2012;18(5):716-25.
30. Robinson JMB, Joel; Cando-Dumancela, Christian; Deshmukh, Sonali; Fickling, Nicole W.; Hawken, Scott; Hayward, Claire; Kuhn, Emma; Lee, Kevin; Liddicoat, Craig; Ramesh, Sunita; Robinson, Kate; Sun, Xin; Breed, Martin F. The ‘Database of Good Things’: a salutogenic approach to microbial and biochemical research. 2025.
31. Fiegel J, Clarke R, Edwards DA. Airborne infectious disease and the suppression of pulmonary bioaerosols. Drug Discov Today. 2006;11(1-2):51-7.
32. Respiratory problems. Australian Journal for General Practitioners. 2012;41:856-60.
33. Chandra D CS. Hypersensitivity Pneumonitis Treasure Island (FL): StatPearls Publishing; 2023 [Updated 2023 Jul 10:[
34. Prussin AJ, II, Garcia EB, Marr LC. Total Concentrations of Virus and Bacteria in Indoor and Outdoor Air. Environmental Science & Technology Letters. 2015;2(4):84-8.
35. Leung NHL. Transmissibility and transmission of respiratory viruses. Nature Reviews Microbiology. 2021;19(8):528-45.
36. Li J, Gao H, Lan C, Nie L, Liu D, Lu X, et al. Plasma air filtration system for intercepting and inactivation of pathogenic microbial aerosols. Journal of Environmental Chemical Engineering. 2023;11(5).
37. Moreno E, Klochok G, García S. Active Versus Passive Flow Control in UVC FILTERs for COVID-19 Containment. Annals of Biomedical Engineering. 2021;49(9):2554-65.
38. Lei H, Xiao S, Cowling BJ, Li Y. Hand hygiene and surface cleaning should be paired for prevention of fomite transmission. Indoor Air. 2020;30(1):49-59.
39. Xiao S, Li C, Zhao F, Lin R, Zhang N, Li Y. A coupled hand and surface hygiene criterion on heterogeneous surface touch networks. Journal of Hazardous Materials. 2024;479.
40. Goss MB, Kroll JH. Organic aerosol formation from 222 nm germicidal light: ozone-initiated vs. non-ozone pathways. Environmental Science: Processes and Impacts. 2024.
41. Snelling WJ, Afkhami A, Turkington HL, Carlisle C, Cosby SL, Hamilton JWJ, et al. Efficacy of single pass UVC air treatment for the inactivation of coronavirus, MS2 coliphage and Staphylococcus aureus bioaerosols. Journal of Aerosol Science. 2022;164.
42. Thi Le VC, Sheraz M, Kang E, Ly HN, Mai HD, Lee WR, et al. Four-in-one multifunctional air filter using copper coordination polymer particle decorated fibre for efficient pathogen removal and indoor air treatment. Process Safety and Environmental Protection. 2022;166:177-88.
43. Bazant MZ, Kodio O, Cohen AE, Khan K, Gu Z, Bush JWM. Monitoring carbon dioxide to quantify the risk of indoor airborne transmission of COVID-19. Flow. 2021;1.
44. Nunayon SS, Mui KW, Wong LT. Mapping the knowledge pattern of ultraviolet germicidal irradiation for cleaner indoor air through the lens of bibliometrics. Journal of Cleaner Production. 2023;391.
45. Zargar B, Sattar SA, Kibbee R, Rubino J, Khalid Ijaz M. Direct and quantitative capture of viable bacteriophages from experimentally contaminated indoor air: A model for the study of airborne vertebrate viruses including SARS-CoV-2. Journal of Applied Microbiology. 2022;132(2):1489-95.
46. Treccani L, Rovetta D, Zanetti G, Gobbi E, Turina M, Lombini M, et al., editors. UVC-Mirror for effective pathogens inactivation in air ducts. E3S Web of Conferences; 2024.
47. Siddiqui R, Khamis M, Ibrahim T, Khan NA. SARS-CoV-2: Disinfection Strategies to Prevent Transmission of Neuropathogens via Air Conditioning Systems. ACS Chemical Neuroscience. 2020;11(20):3177-9.
48. Kolarž P, Ilić AŽ, Janković M, Janićijević A, Trbovich AM. Estimating aerosol particle removal in indoor air by ion-enhanced deposition. Journal of Aerosol Science. 2023;173.
49. Szeto W, Yam WC, Huang H, Leung DYC. The efficacy of vacuum-ultraviolet light disinfection of some common environmental pathogens. BMC Infectious Diseases. 2020;20(1).
50. Xiao L, Du Z. Effects of Evaporative Cooling Air Conditioning on Classroom Pollutants and Thermal Environment. Environ Health Insights. 2022;16:11786302221113995.
51. International S. Ventilation and indoor air quality Lonsdale, South Australia: Seeley International; 2022
52. Paschold H, Li W-W, Morales H, Walton J. Laboratory study of the impact of evaporative coolers on indoor PM concentrations. Atmospheric Environment. 2003;37(8):1075-86.
53. Long CM, Suh HH, Koutrakis P. Characterization of indoor particle sources using continuous mass and size monitors. J Air Waste Manag Assoc. 2000;50(7):1236-50.
Latest edition
See everything from the latest edition of Ecolibrium, AIRAH’s official journal.